As energy storage becomes an increasingly integral part of a renewables-based system, interest in and discussion around non-lithium (and non-pumped hydro) technologies increases. A team of experts from CENELEST, a joint research venture between the Fraunhofer Institute for Chemical Technologies and the University of New South Wales take a deep dive into redox flow batteries.
In the last 15 years, the increase in renewable energy sources such as photovoltaic and wind energy has accelerated significantly. At the same time, manufacturing and installation costs, especially for PV systems, have fallen significantly, making this energy source one of the cheapest and cleanest forms of energy, depending on the location. With the increase of fluctuating renewable energies in an electrical grid, the need for compensation possibilities at times when renewable energies are not available increases [1]. One possibility is the use of electrochemical energy storage such as lithium-ion, lead-acid, sodium-sulphur or redox-flow batteries. Additionally, combinations of hydrogen electrolysis and fuel cells can be used [2]. Batteries can be adapted in a flexible and decentralised manner depending on the respective requirements and are scaleable from a few kW/kWh for e.g. domestic storage up to systems of several MW/MWh for grid storage. The different types of electrochemical energy storage systems have different physical/chemical properties, which affect the cost of the system. It is important to note that the cost of the storage system over its lifetime (levelised cost of storage – LCOS) is a critical factor used in selecting the most suitable system for a particular application [3]. For example, the investment costs for lead-acid batteries are significantly lower than for all other technologies, but the service life is very short.
Enjoy 12 months of exclusive analysis
- Regular insight and analysis of the industry’s biggest developments
- In-depth interviews with the industry’s leading figures
- Annual digital subscription to the PV Tech Power journal
- Discounts on Solar Media’s portfolio of events, in-person and virtual
Technologies with similar investment costs at higher lifetimes result in a lower levelised cost of storage, but to be precise additional factors such as recycling, energy efficiency and maintenance costs have to be considered. A battery with a high efficiency, low recycling effort, low investment and maintenance costs and great freedom of scalability to meet the requirements of the application would be an ideal system. In electrical networks there are different storage time requirements: short-term storage, medium-term storage and long-term storage. The shorter the storage time, the more suitable are physical storage devices such as capacitors. Batteries are suitable for applications ranging from a few minutes to several hours. In addition, mass storage systems such as electrochemical hydrogen generation (power-to-gas) are particularly suitable for long-term storage of several weeks.
Redox flow principles
All electrochemical energy storage systems convert electrical energy into chemical energy when charging, and the process is reversed when discharging. With conventional batteries, the conversion and storage take place in closed cells. With redox flow batteries, however, the conversion and storage of energy are separated [4]. Redox flow batteries differ from conventional batteries in that the energy storage material is conveyed by an energy converter. This requires the energy storage material to be in a flowable form. This structure is similar to that of fuel cells, whereby in redox flow batteries, charging and discharging processes can take place in the same cell. Redox flow batteries thus have the distinguishing feature that energy and power can be scaled separately. The power determines the cell size or the number of cells and the energy is determined by the amount of the energy storage medium. This allows redox flow batteries to be better adapted to certain requirements than other technologies. In theory, there is no limit to the amount of energy and often the specific investment costs decrease with an increase in the energy/power ratio, as the energy storage medium usually has comparatively low costs. Figure 1 shows the general operating principle of redox flow batteries. The energy conversion takes place in an electrochemical cell which is divided into two half cells. The half cells are separated from each other by an ion-permeable membrane or separator, so that the liquids of the half cells mix as little as possible. The separator ensures a charge balance between positive and negative half cells, ideally without the negative and positive active materials coming into direct contact with each other. In fact, however, separators are not perfect so some cross-over of the active materials always occurs and this leads to the self-discharge effect.
In a single cell there is always one positive and one negative half-cell. The electrochemical reactions for charging and discharging take place at the electrodes of the half-cells. The electrodes are the phase transitions of ionic and electronic conductors. In redox flow batteries, the electrodes should not participate in the reactions for energy conversion and should not cause any further side reactions (e.g. undesirable gas formation). Most redox flow batteries are therefore based on carbon electrodes.
The difference between the voltages of the positive electrode and the negative electrode is the cell voltage and is between 0.5 and 1.6V in aqueous systems. During the charging process, ions are oxidised at the positive electrode (electron release) and reduced at the negative electrode (electron uptake). This means that the electrons move from the active material of the positive electrode to the active material of the negative electrode. When discharging, the process reverses and energy is released. The active materials are redox pairs, i.e. chemical compounds that can absorb and release electrons.
In redox flow batteries, the energy storage medium is often referred to as an electrolyte. However, there are redox flow batteries that use a gas that is not an electrolyte (e.g. H/Br-RFB) as with hydrogen. As with all other aqueous batteries, aqueous energy storage media from redox flow batteries are also subject to water limitations. In case of too high voltages or more precisely too high or too low half-cell potentials, the water is decomposed into its components, hydrogen and oxygen. The generation of hydrogen in particular is often present as a very small but undesirable side reaction and causes a charge carrier imbalance between positive and negative half-cells, which leads to a slow loss of capacity. Due to the flowability of the energy storage medium, the reaction products that would normally remain in the half-cell can be transported out of the cell and stored in separate tanks thus allowing the capability for a higher capacity than that attainable with conventional batteries.
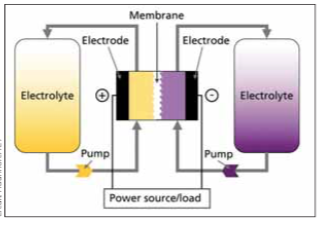
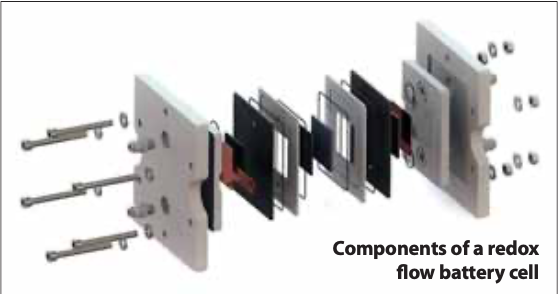
Hybrid redox flow batteries
In redox flow batteries there are normally no phase transitions with solid active materials as with other batteries. This can significantly increase battery life because no lattice structures have to be rebuilt each time the battery is charged or discharged, and all materials are in a solution. The best known representative of redox flow batteries today is the vanadium redox flow battery. However, there are also flow batteries in which solids are deposited and dissolved at one or both electrodes. A typical representative is the zinc/bromine redox flow battery and patented in 1885 by Charles Bradley [5]. Such batteries are called hybrid redox flow batteries. In contrast to redox flow batteries, power and energy are not separately scalable, as the amount of possible solids deposition is limited by the cell geometry.
Hybrid redox flow batteries also usually have two electrolyte circuits like conventional redox flow batteries, but too much active material would lead to a too-thick layer of solids in the half-cell and thus to clogging of the fluidic system or to so-called dendrites, which are uneven deposits and can lead to short circuits through the separator. As with all other batteries, however, the power density decreases with the layer thickness of the half cells. For this reason, the space for the deposition and thus the layer thickness is optimised with regard to the power density and usually leads to storage times of approximately 4-8 hours. The advantages of the Zn/Br redox flow battery are the low costs of the active materials, zinc and bromine, and the high energy density of approximately 70-80Wh/litre. The disadvantages are above all the use of bromine and the relatively short cycle life with several thousand charging and discharging cycles. Elementary bromine is produced when charging the battery at the positive electrode. In order to reduce the risk potential and self-discharge, organic complexing agents are added to the energy storage medium to bind the bromine and prevent it from escaping [6]. The complexing agent is relatively expensive and the subject of research to reduce battery costs. The used redox pair Br/Br- has a very high reaction speed and is ideal for batteries from an electrochemical point of view. In the 1970s, Exxon and General Electric launched relevant commercialisation efforts in the USA and led to stack concepts, materials and production technologies that are still relevant today.
Later, starting in the 1980s, commercialisation efforts were made mainly by ZBB Corp. Australia, which developed modular multi-megawatt battery systems but stopped working a few years ago. In the 1980s, developments were also made for use in electric vehicles, primarily by the Austrian companies SEA and Powercell. The batteries were used experimentally in various commercial vehicles and buses. A vehicle with a Powercell battery finished first in the EV Division of the 1994 and 1995 World Clean Air Vehicle Rallies in California [7] Today only one company is selling Zn/Br-RFBs.
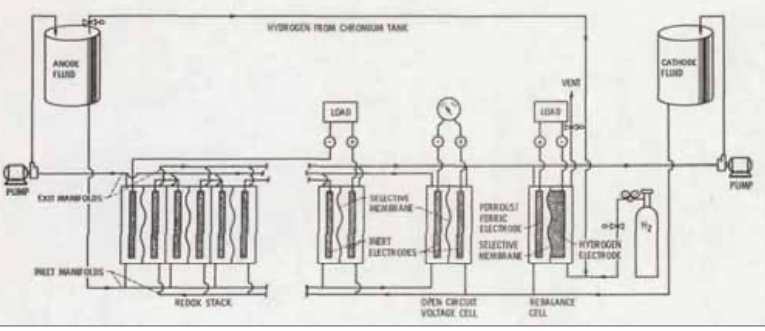
The first redox flow batteries were patented by Kangro in 1949 [8]. Kangro’s motivation was, at that time, storing energy for wind and tidal power plants. Kangro’s patent includes redox flow batteries based on the elements chromium, iron, titanium and chlorine. None of these systems have ever made it into commercialisation due to toxicity or technical problems. Pieper, a PhD student of Kangro, worked again on redox flow batteries in his doctoral thesis at the end of the 1950s [9, 10]. Pieper systematically investigated the potential of many inorganic active materials for applications in redox flow batteries. It is interesting to note that he also included vanadium in his considerations, but based on literature searches in which side reactions, in particular hydrogen development at platinum electrodes, were reported, he ruled out suitability. Pieper later used carbon electrodes, however, that standard material, which is also used today for vanadium redox flow batteries, in his experiments with other active materials. A vanadium redox flow battery would have been possible with his experiments as early as the 1950s. However, Pieper favoured a titanium/iron redox flow battery, which was later further developed by NASA [11].
The Ti/Fe-RFB uses Fe2+/Fe3+ as negative and Ti3+/TiO2+ as positive redox pair. The reactions of Fe2+/Fe3+ are very fast, but the reactions of Ti3+/TiO2+ are much slower, which makes the energy efficiency and power density relatively low. The Ti/ Fe-RFB has the disadvantage of a low cell voltage of about 0.8V and a low concentration of active materials. The maximum achievable energy density is thus approximately 10Wh/L and practically much lower. For these reasons the Ti/Fe-RFB has never been successful.
With the beginning of the first oil price crisis in 1973, a rethinking of the energy supply began. Investments in regenerative energy sources and the necessary research and development of storage systems for fluctuating energy producers led to the development of the iron/chromium redox flow battery at NASA by Thaller [12]. Thaller was also the first to use the term “Redox Flow Cell”. In the Fe/Cr-RFB, as in the Ti/Fe-RFB, the redox pair Fe2+/Fe3+ is used, but on the positive electrode. As already mentioned above, the reaction rate of the redox pair is high and thus are the achievable power density and energy efficiency. Iron is also an extremely inexpensive material for energy storage. By far the greatest challenges occur with the reactions of chromium ions at the negative electrode. The redox reactions of Cr2+/Cr3+ are very slow and are close to hydrogen generation, so the efficiency of the reactions is very low. NASA’s work was therefore primarily concerned with these reactions and their acceleration by catalysts and the suppression of hydrogen formation by inhibitors. Prior to this, however, there was again a screening of possible candidates as active materials for redox flow batteries. Again, vanadium was considered on a theoretical basis, but ultimately due to the cost was not further studied. Iron and chromium were selected because of potentially low costs [14]. NASA’s work led to a demonstration system with an output of 1kW/13kWh as a domestic storage system coupled with a PV system [15] and lasted until around the mid-1980s. With the lowering of crude oil prices, the general interest in renewable energies and storage facilities decreased so that no commercialisation took place. It was not until the mid-2000s that various companies attempted to commercialise Fe/Cr-RFBs again, but these were discontinued.
In 1981, Hruska and Savinell published an article about a hybrid redox flow battery that only uses iron as an active material [16]. The motivation was the use of an energy storage material that was as inexpensive as possible, which is almost unsurpassable with iron. One kilogram of iron corresponds to approximately 500Wh, or 1kWh would cause approximately US$5 in active material costs. The Fe/Fe-RFB uses the soluble redox pair Fe2+/Fe3+ at the positive electrode but the redox pair Fe/Fe2+ at the negative electrode just like the two iron-based RFBs discussed above.
The initial solution is a relatively simple and widely available Fe(II) salt solution, similar to that used on a large scale in wastewater treatment. Solid and elemental iron is deposited on the negative electrode during charging and dissolved again during discharge. The challenges with this battery are the hydrogen generation at the negative electrode and the low reaction rate of Fe/Fe2+ at the negative electrode.
The generation of hydrogen leads to a significant loss of capacity, which can, however, be prevented or reversed by appropriate measures. The low reaction rates result in low efficiency, which can be increased by operating at elevated temperatures between 50-80°C. The low reaction rates result in low efficiency. The energy efficiency is approx. 60-70%, but it should be noted that energy efficiencies must be considered in connection with the application. The potentially low investment costs of the battery, together with compensation for losses from low-cost renewable energy, can result in a lower levelised cost of energy than other storage technologies. In the last 10 years, research and commercialisation activities have increased, albeit at a very low level.
Vanadium redox flow batteries
At about the same time as NASA’s developments came to an end, the University of New South Wales conducted investigations into vanadium ions as an active material for redox flow batteries. Maria Skyllas-Kazacos et al finally found the possibility of using four different oxidation states on carbon electrodes in fundamental electrochemical investigations [17, 18] and later as battery experiments [19].
In the following years, almost all aspects of the vanadium redox flow battery (VRFB) were investigated at the UNSW. Efficient manufacturing processes were developed from inexpensive and widely available raw materials for the active material, the electrochemical and chemical basics were investigated, the energy density was increased through the use of concentrated solutions and stabilising agents, inexpensive separators and membrane modification methods were investigated and the power density was increased through electrode treatment processes. Stack cost reduction was also achieved by the development of an inexpensive conducting plastic bipolar electrode substrate that can be welded to the cell flow frame. In 1989, the work led to a battery with 1kW power and its performance was reported in 1991 [20]. This was followed by the installation of a 15kWh VRFB by the UNSW team in a Solar Demonstration House in Thailand and the licensing of the UNSW patents and technology to Mitsubishi Chemicals/ Kashima Kita Power Corporation (MC/ KKEPC), Japan in 1994. This was followed by a five-year R&D collaboration programme between UNSW and MC/ KKEPC that led to the installation and testing of a 200kW/800kWh VRFB at Kashima Kita in 1995. In the following years, more and more work was carried out by other research groups and further commercialisation trials were carried out by UNSW’s licensees. Particularly from the 2000s onwards, interest in research and industry increased sharply.
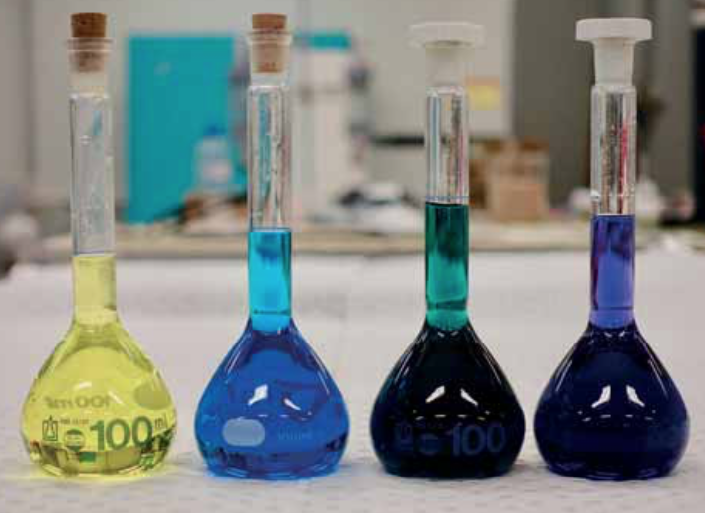
Today, the vanadium redox flow battery is without doubt the best investigated and most installed redox flow battery. Numerous patents and publications cover almost all aspects of the vanadium redox flow battery (VRFB). The VRFB uses only vanadium as an active material in three different oxidation states. The redox pair VO2+/VO2+ at the positive electrode and the redox pair V2+/V3+ at the negative electrode. The use of the same ions in the positive and negative electrolytes permits relatively high concentrations of active material. This allows the classical VRFB to keep up to approximately 1.8mol/L vanadium in solution and thus achieve maximum energy density of up to approximately 38Wh/L. Vanadium is a relatively frequently occurring metal that is enriched as a by-product during the combustion of fossil fuels. Its main application is as an alloying metal for steel production. By using vanadium ions in solution, potentially very high cycle lifetimes can be achieved, as no complex phase transitions and new phase build- ups are necessary.
A further advantage is the simple recyclability of the batteries. Due to the high content of vanadium in the liquid electrolyte, the vanadium can easily be reintegrated into process chains and the existing value reused. The energy storage solution consists primarily of vanadium sulphate in a diluted (2mol/L) sulphuric acid containing a low concentration of phosphoric acid and is therefore roughly comparable to the acid of lead/acid batteries. The energy density is limited by the concentration of the pentavalent + VO2.
Unfortunately, pentavalent vanadium ions have a tendency to react with each other, which leads to the formation of larger molecules which precipitate as solids and can thus damage the system. The reaction depends on the temperature and the concentration of VO2+ (state of charge), but is also a function of the proton concentration. Increasing the acid concentration increases the stability of VO2+, but this reduces the solubility of the V(II), V(III) and V(IV) sulphates. The vanadium and total sulphate concentrations are thus set at around 1.8 and 4.2mol/L respectively in order to achieve an acceptable operating temperature range. With a high state of charge and elevated temperature, the tendency to form solids in the positive half-cell increases, which is why the electrolyte temperature is usually limited to a maximum of 40°C. To minimise the risk of precipitation of the other species at low temperatures, a lower limit of 10°C is usually recommended. Alternatively, the SOC limits can be adjusted to handle temperatures outside this range.
Like all other RFBs, VRFBs also have a battery management. A battery management ensures optimum and safe conditions for battery operation. Often a heat management system is integrated to avoid too high or too low temperatures. The classic VRFB has undergone several further developments. First, the V/Br-RFB called Gen 2 was developed by Skyllas- Kazacos et al. at the UNSW [21].
By using the fast redox pair Br2/Br-, the objectives were a higher energy density and a better temperature stability, as well as possibly higher energy efficiency. Gen 3 was developed at the Pacific North West National Laboratory (PNNL) and uses a mixture of hydrochloric acid and sulfuric acid as solvent for the vanadium ions [22]. This increases temperature stability and energy density up to 50Wh/L. A disadvantage is the internal formation of chlorine gas in the system, which has a higher demand on the stability of materials. Vanadium/oxygen cells are referred to as Gen 4, in which vanadium ions are oxidised by oxygen (e.g. from the air) during discharge and energy can thus be generated. The process is reversed in the charging process. Theoretically up to 150Wh/L energy density can be achieved [23]. Today’s VRFBs range from a few KW/KWh to several hundred MW/MWh. The applications range from home storage to industrial plants as large storage units in the grid. Several companies sell VRFBs today in different size classes.
Commercialisation and ongoing research
From the 2000s onwards, the number of scientific publications and commercialisation efforts for RFB types other than those mentioned here increased significantly. Basically, there are many possible combinations for inorganic RFBs. By 2015, there were about 78 different types of RFB, but only a few of them have or will ever have commercial relevance [4]. Of the many different combinations, Pb/Pb- and Zn/ Ce- and H/Br-RFBs were the most studied in addition to the ones mentioned above [24, 25, 26]. The major challenges for new aqueous inorganic RFBs are above all the electrochemical window limited to a maximum of 2.1V, in which the redox pairs can function largely without hydrogen and oxygen formation, side reactions of the redox pairs with the solvent water and costs of the active materials. Especially the limitation of the voltage by the use of water led to investigations on the use of non-aqueous alternative solutions and redox pairs. The maximum possible voltage correlates with the maximum possible energy of an RFB. Doubling the voltage to 4V, as with lithium-ion batteries, doubles the maximum possible energy density. However, it should be noted that the cell resistance significantly determines the real energy density and often only very low energy densities can be achieved with very high cell resistances. Liu et al at the University of Michigan trialled the approach of using organic solvents to increase the energy density for the first time in 2009 with a vanadium-based organic acetylacetonate complex in acetonitrile [27]. Cell voltage versus aqueous inorganic VRFB increased from 1.6V to 2.2V.
The same group also showed potential for non-aqueous chromium and manganese-based RFBs [28, 29]. In 2011 also, the concept of a lithium-based RFB attracted attention [30]. As with conventional lithium-ion batteries, solids were used for the anode and cathode. To make the active materials flowable, they were used as suspensions in an organic liquid. Suspensions are mixtures of solid and liquid components. The authors expected energy densities of up to 250Wh/L. In the following years many different concepts of Li-RFBs have been investigated, but it is not known that commercialisation efforts have ever been made. It is likely that the costs associated with low cycle life and low current density are the reasons why there has been little work in the field in recent years.
The first completely organic RFB, i.e. organic redox pairs and organic solvent, was presented in 2011 by Li et al [31]. The advantage of a fully organic battery lies in the potentially low cost of organic active materials, their high availability and ease of disposal. However, organic solvents have an increased risk potential due to their flammability, and only low power densities can be achieved due to their low conductivity. These reasons in turn led to a focus on aqueous organic RFBs. The first organic RFB based on water as solvent and organic redox pairs was published in 2014 by Yang et al [32]. The authors used modified quinone and anthraquinone as active materials, substance classes that also occur as natural dyes. In the following years the research activity in the field of organic, especially aqueous RFBs increased significantly.
The multitude of possibilities for organic active materials is considerably higher than for inorganic RFBs. However, there are also limits, so that the molecules must not be arbitrarily large, because otherwise they would have a too high mass and thus low energy density. However, organic molecules also offer the possibility of several electron transitions. In the case of inorganic active materials, one electron transition or two electron transitions are usually used. A doubling of the number of electron transitions leads to a doubling of the capacity (Ah) and with the same properties a doubling of the energy density.
However, organic RFBs can potentially have up to six and perhaps more electron transitions. However, the reactions of organic active materials are often very complex and side reactions can lead to a small but continuous loss of capacity. The transfer of the results from research is not easy, since often only small concentrations and quantities of active materials are used and the properties in real batteries can be completely different. These can be e.g. deposition effects on electrodes which reduce the power density or limit the capacity or a gradual destruction of the active materials. Although the advantages are clear, there is still a long way to go for a practicable use of organic RFBs.
Sunlight can be stored directly in chemical energy by means of photocatalytic reactions. The best-known processes are natural photosynthesis and the artificial photolysis of water into hydrogen and oxygen. The objectives
are to reduce the number of conversions through more compact systems and to increase the compactness of the systems. In 2014, Liu et. al. showed an approach in which a VRFB with a suitable catalyst and a transparent positive electrode can use light with a yield of up to 12% directly to charge the battery [33]. At present, however, these investigations are still very much basic research.
RFBs have experienced highs and lows throughout their history. The reason for this is that the idea was usually ahead of its time and the intended use, i.e. the stationary storage of energy, was not given to the extent required to develop competitive products. With the significant increase in renewable energy in the last 15 years, however, the situation has changed significantly. It cannot be foreseen that lithium-ion batteries will be the technology that will take over mobile and stationary tasks at low cost. Lithium- ion batteries currently lead to social problems (cobalt mining in the Congo), hazards (fire) and problems with the very expensive recycling (environmental aspects) of the many hazardous substances (cobalt, nickel, organic electrolytes). Lithium-ion batteries do not seem to be a sustainable and green technology currently. The demand for stationary storage facilities is growing every year and so is the demand for electric mobility. The costs of RFBs, especially VRFB and Zn/Br-RFB, have also fallen significantly over the last 10 years. These price reductions, however, still took place through the installation of comparatively few storage devices, mainly for demonstration plants. With an increase in the number of units and thus possible economies of scale and an optimisation of production towards mass production, further significant reductions in RFB’s costs can be achieved.
The Fraunhofer ICT and University of New South Wales are working together as an alliance to intensify research activities in the field of electrochemical energy storage and to establish a joint international research centre – CENELEST – at UNSW. The aim is to strengthen expertise in redox flow batteries and to develop other types of batteries and fuel cells in order to cover the entire range of electrochemical energy storage needs for renewable energy.
This article first appeared in Energy-Storage.news' 'Storage & Smart Power' – a dedicated section of Solar Media's quarterly technical journal PV Tech Power (Volume 21). Download the full magazine as a PDF for free, here (subscription details required). You can also download this article and the rest of 'Storage & Smart Power' as standalone PDFs in the 'Resources' section of this site.
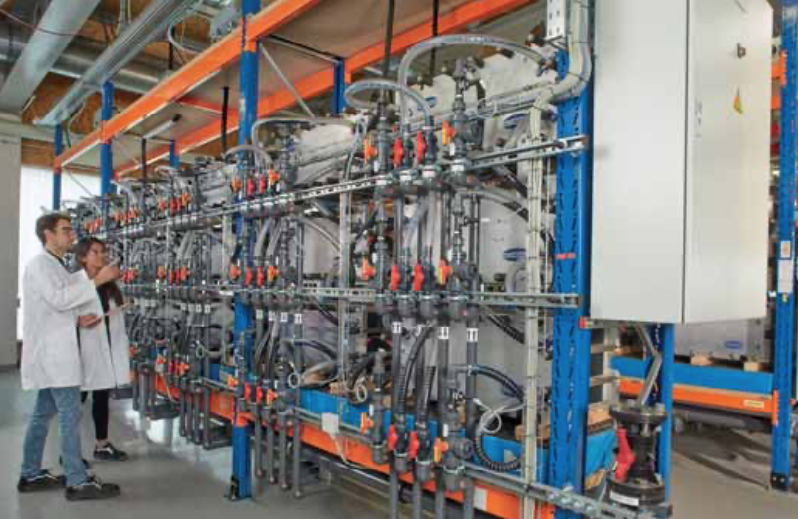
References
[1] Amirante, R.; Cassone, E.; Distaso, E.; Tamburrano, P. Overview on recent developments in energy storage: Mechanical, electrochemical and hydrogen technologies. Energy Conversion and Management 2017, 132, 372–387, doi:10.1016/j.enconman.2016.11.046.
[2] Cebulla, F.; Haas, J.; Eichman, J.; Nowak, W.; Mancarella, P. How much electrical energy storage do we need?: A synthesis for the U.S., Europe, and Germany. Journal of Cleaner Production 2018, 181, 449–459, doi:10.1016/j.jclepro.2018.01.144.
[3] Schmidt, O.; Melchior, S.; Hawkes, A.; Staffell, I. Projecting the Future Levelized Cost of Electricity Storage Technologies. Joule 2019, 3, 81–100, doi:10.1016/j.joule.2018.12.008.
[4] Noack, J.; Roznyatovskaya, N.; Herr, T.; Fischer, P. The Chemistry of Redox-Flow Batteries. Angew. Chem. Int. Ed. 2015, 54, 9776-9809, doi:10.1002/anie.201410823.
[5] C.S. Bradley. Secondary Battery, US Patent 312802, 1885.
[6] Jeon, J.-D.; Yang, H.S.; Shim, J.; Kim, H.S.; Yang, J.H. Dual function of quaternary ammonium inZn/Br redox flow battery: Capturing the bromine and lowering the charge transfer resistance.Electrochimica Acta 2014, 127, 397–402, doi:10.1016/j.electacta.2014.02.073.
[7] Rand, D., A., J.; Woods, R.; Dell, R., M. Batteries for Electric Vehicles. Research Studies Press Ltd.,1998
[8] W. Kangro. Verfahren zur Speicherung von elektrischer Energie. German Patent 914264, 1949
[9] Heinz Pieper. Zur Frage der Speicherung von elektrischer Energie in Flüssigkeiten. Dissertation; Technische Hochschule Braunschweig, Braunschweig, 1958.
[10] W. Kangro, H.P. Zur Frage der Speicherung von elektrischer Energie in Flüssigkeiten. Electrochimica Acta (1962) Vol. 7, 435-448 1962.
[11] M.A. Reid, R.F.G. Factors affecting the open circuit voltage and electrode kinetics of some irontitanium redox flow cells. NASA TMX-73669, National Aeronautics and Space Administration,U.S. Dept. Of Energy, 1977 1977.
[12] L.H. Thaller. Electrically rechargeable redox flow cells. Proc. 9th Intersoc. Energy Conv. Eng.Conf., San Francisco, CA, 26-30 August, 1974; NASA TM X-71540, p. 924 1974.
[13] N.H. Hagedorn, L.H.T. Design Flexibility of Redox Flow Systems [for energy storage applications] NASA-TM-82854; E-1223; NAS 1.15:82854; DOE/NASA/12726-16, 1982.
[14] J.D. Giner, K.C. Advanced screening of electrode couples NASA-CR-159738; DOE/NASA/0794- 80/1, 1980. http://ntrs.nasa.gov/archive/nasa/casi.ntrs.nasa.gov/19800014289_1980014289.pdf.
[15] Hagedorn, N.H. NASA Redox Storage System Development Project NASA-TM-83677;E-2125; NAS 1.15:83677, 1984. http://ntrs.nasa.gov/archive/nasa/casi.ntrs.nasa.gov/19850004157_1985004157.pdf.
[16] Hruska, L. W.; Savinell, R. F. Investigation of Factors Affecting Performance of the Iron-RedoxBattery. J. Electrochem. Soc. 1981, 128, 18, doi:10.1149/1.2127366.
[17] Sum, E.; Skyllas-Kazacos, M. A study of the V(II)/V(III) redox couple for redox flow cell applications. Journal of Power Sources 1985, 15, 179–190, doi:10.1016/0378-7753(85)80071-9. [18] Sum, E.; Rychcik, M.; Skyllas-Kazacos, M. Investigation of the V(V)/V(IV) system for use in the positive half-cell of a redox battery. Journal of Power Sources 1985, 16, 85–95, doi:10.1016/0378-7753(85)80082-3.
[19] Skyllas‐Kazacos, M.; Rychcik, M.; Robins, R.G.; Fane, A.G.; Green, M.A. New All‐Vanadium Redox Flow Cell. Journal of The Electrochemical Society 1986, 133, 1057–1058, doi:10.1149/1.2108706. [20] Skyllas-Kazacos, M.; Kasherman, D.; Hong, D.R.; Kazacos, M. Characteristics and performance of 1 kW UNSW vanadium redox battery. Journal of Power Sources 1991, 35, 399–404, doi:10.1016/0378-7753(91)80058-6.
[21] M. Skyllas-Kazacos. Novel vanadium chloride polyhalide redox flow battery. Journal of PowerSources 124 (2003) 299-302 2003.
[22] Vijayakumar, M.; Wang, W.; Nie, Z.; Sprenkle, V.; Hu, J. Elucidating the higher stability of vanadium(V) cations in mixed acid based redox flow battery electrolytes. Journal of Power Sources 2013, 241, 173–177, doi:10.1016/j.jpowsour.2013.04.072.
[23] Risbud, M.; Menictas, C.; Skyllas-Kazacos, M.; Noack, J. Vanadium Oxygen Fuel Cell Utilising High Concentration Electrolyte. Batteries 2019, 5, 24, doi:10.3390/batteries5010024.
[24] Krishna, M.; Fraser, E.J.; Wills, R.G.A.; Walsh, F.C. Developments in soluble lead flow batteries and remaining challenges: An illustrated review. Journal of Energy Storage 2018, 15, 69–90, doi:10.1016/j.est.2017.10.020.
[25] Leung, P.K.; Ponce-de-León, C.; Low, C.T.J.; Shah, A.A.; Walsh, F.C. Characterization of a zinc–cerium flow battery. Journal of Power Sources 2011, 196, 5174–5185, doi:10.1016/j.jpowsour.2011.01.095.
[26] Yeo, R.S.; Chin, D.‐T. A Hydrogen‐Bromine Cell for Energy Storage Applications. Journal of TheElectrochemical Society 1980, 127, 549–555, doi:10.1149/1.2129710.
[27] Liu, Q.; Sleightholme, A.E.S.; Shinkle, A.A.; Li, Y.; Thompson, L.T. Non-aqueous vanadium acetylacetonate electrolyte for redox flow batteries. Electrochemistry Communications 2009, 11, 2312–2315, doi:10.1016/j.elecom.2009.10.006.
[28] Liu, Q.; Shinkle, A.A.; Li, Y.; Monroe, C.W.; Thompson, L.T.; Sleightholme, A.E.S. Non-aqueous chromium acetylacetonate electrolyte for redox flow batteries. Electrochemistry Communications 2010, 12, 1634–1637, doi:10.1016/j.elecom.2010.09.013.
[29] Sleightholme, A.E.S.; Shinkle, A.A.; Liu, Q.; Li, Y.; Monroe, C.W.; Thompson, L.T. Non-aqueous manganese acetylacetonate electrolyte for redox flow batteries. Journal of Power Sources 2011, 196, 5742–5745, doi:10.1016/j.jpowsour.2011.02.020.
[30] Duduta, M.; Ho, B.; Wood, V.C.; Limthongkul, P.; Brunini, V.E.; Carter, W.C.; Chiang, Y.-M. Semi-Solid Lithium Rechargeable Flow Battery. Adv. Energy Mater. 2011, 1, 511–516, doi:10.1002/aenm.201100152.
[31] Li, Z.; Li, S.; Liu, S.; HUANG, K.; Fang, D.; Wang, F.; Peng, S. Electrochemical Properties of an All-Organic Redox Flow Battery Using 2,2,6,6-Tetramethyl-1-Piperidinyloxy and N-Methylphthalimide. Electrochem. Solid-State Lett 2011, 14, A171-A173, doi:10.1149/2.012112esl.
[32] Yang, B.; Hoober-Burkhardt, L.; Wang, F.; Surya Prakash, G.K.; Narayanan, S.R. An Inexpensive Aqueous Flow Battery for Large-Scale Electrical Energy Storage Based on Water-Soluble Organic Redox Couples. J. Electrochem. Soc 2014, 161, A1371-A1380, doi:10.1149/2.1001409jes.
[33] Wei, Z.; Liu, D.; Hsu, C.; Liu, F. All-vanadium redox photoelectrochemical cell: An approach to store solar energy. Electrochemistry Communications 2014, 45, 79–82, doi:10.1016/j. elecom.2014.05.018.